D. Terwagne
G. Delon
N. Vandewalle
H. Caps
S. Dorbolo
GRASP, Opto-fluidique Département de Physique B5
Université de Liège B-4000
Liège, Belgium
At least once in one's life, one spends hours blowing bubbles out of a soapy water film entrapped in a frame. On the other hand, very few people spend hours making antibubbles whereas it is very easy to generate. An antibubble is the inverse of a bubble. Who would expect that something spectacular may result when soapy water is poured on a pool of the same soapy water? By adjusting the flow, it is possible to entrain a thin air film that is able to imprison a 10 mm soapy water globule beneath the pool surface. Numerous tricks exist on the web [1]. Their scientific discovery dates as from 1932 [2] but developments can be only found in the last decade [3,4,5]
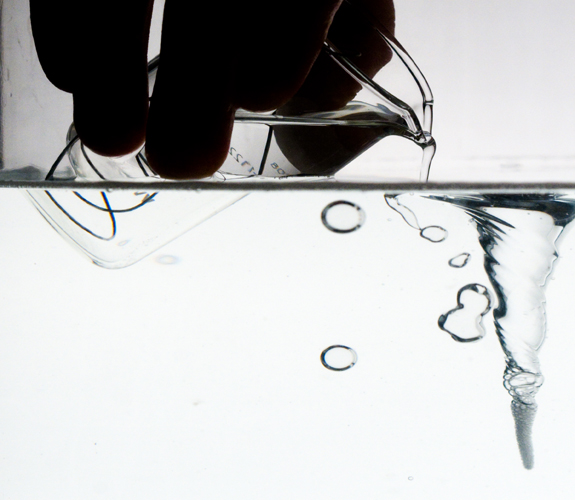
In the present work, antibubbles are precipitated in a whirlpool. A whirl is a singularity in the liquid and attracts the objects that venture to close. This latter effect is due to the morphology of the flow around the vortex. The speed of the flow varies rapidly close to the eye. An object that approaches the eyewall is submitted to large constraint and (if it is deformable) the object is modified by the stress.
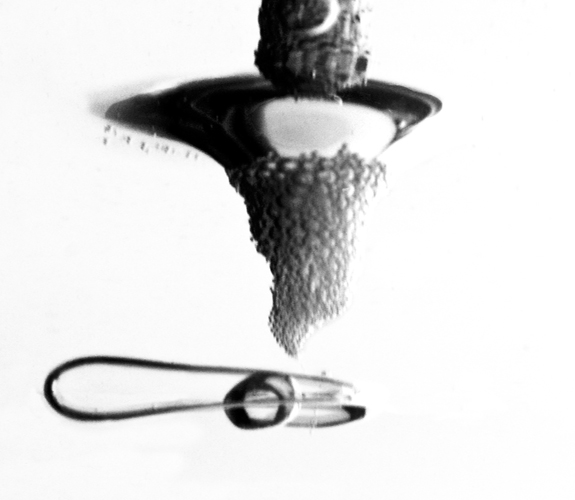
According to the antibubble size, different scenarios have been encountered. For example, the antibubble may be vertically expanded before explosion. Another spectacular example is observed when the antibubble wound around the vortex like a serpentine forming an "anti-spaghetti".
S. Dorbolo is supported by F.R.S.-FNRS. Part of this work has been helped by exchanges through COST P21 "Physics of droplet" network (ESF).
References
[1] see http://www.antibubble.com, http://www.youtube.com/stephanedorbo and also C.L. Stong, Scientific American, 230, 116 (April 1974).
[2] W. Hughes and A.R. Hughes, Nature 129, 59 (1932).
[3] S. Dorbolo, E. Reyssat, N. Vandewalle, and D. Quéré, Europhys. Lett. 69, 966 (2005).
[4] S. Dorbolo, H. Caps, and N. Vandewalle, New J. Phys. 5, 161 (2003).
[5] P. Geon Kim and H. Stone, Europhys. Lett. 83, 54001 (2008).
Reporters and Editors
Reporters can freely use these images. Credit: Jens Kasten, Christoph Petz, Ingrid Hotz, Gilead Tadmor, Bernd R. Noack, Hans-Christian Hege.