Christophe Wylock, Sam Dehaeck, Alexey Rednikov, Pierre Colinet
Chemical Engineering Dept, Free University of Brussels
One step in the production of baking soda consists of absorbing carbon dioxide into a liquid mixture. When the carbon dioxide enters this liquid, a chemical reaction occurs, capturing the carbon dioxide into a baking soda molecule. As this is heavier than the starting liquid, these molecules sink to the bottom of the mixture, giving more room for other carbon dioxide molecules to be captured. In the image, two of these downward jets are visualized by means of digital holographic interferometry.
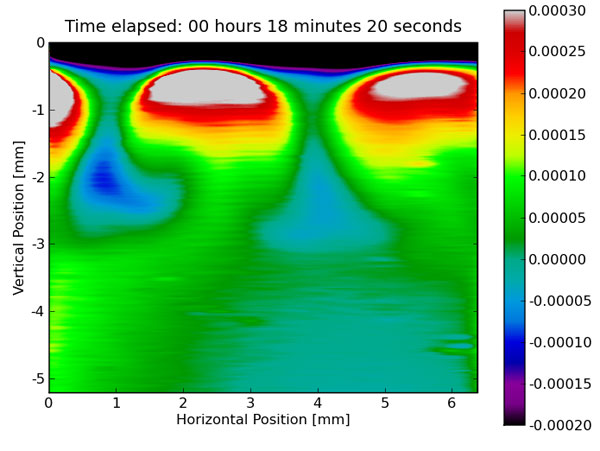
The emission of carbon dioxide into the atmosphere of typical chemical industries can be reduced by absorbing carbon dioxide into optimized liquid mixtures. When the carbon dioxide enters these liquids, a chemical reaction occurs, capturing the carbon dioxide into a new chemical molecule. As the newly formed molecule is heavier than the starting liquid, these molecules sink to the bottom of the mixture, giving more room for other carbon dioxide molecules to be captured. In the image, multiple trains of these new molecules that are transported downwards are visualized by means of digital holographic interferometry.
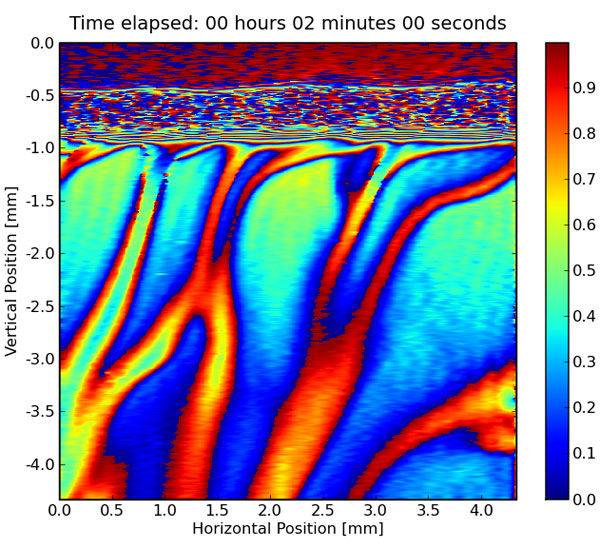
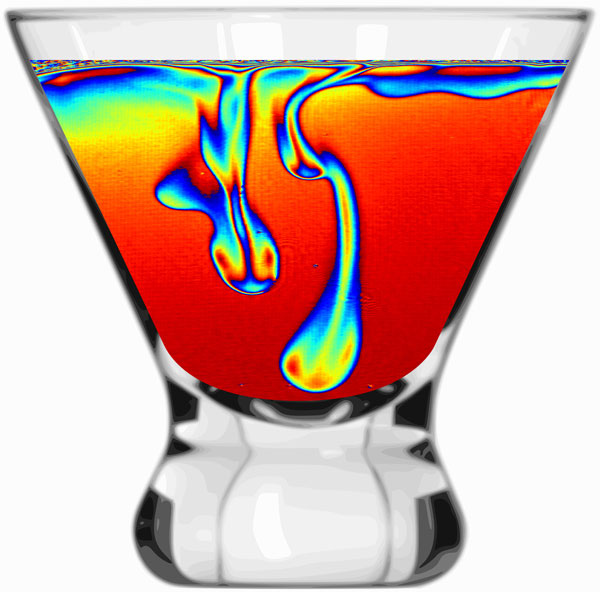
When a mixture of water and alcohol is evaporating, the alcohol will evaporate faster. As a result, the liquid at the surface will contain relatively more water than the rest of the cocktail. This corresponds to a heavier liquid and therefore it sinks to the bottom of the glass. In the image, such sinking, water-rich structures are visualized by means of digital holographic interferometry.
This work was supported by F.R.S.- F.N.R.S. (Belgian National Fund for Scientific Research); CIMEX-PRODEX Programme managed by the European Space Agency in collaboration with the Belgian Federal Science Policy Office, and by the ARCHIMEDES (ARC 04/09-308) project funded by the Communauté Française de Belgique.
References
The image Carbon Dioxide-Absorbing Aqueous Brine appeared in "Chemo-hydrodynamical instability created by CO2 absorption in an aqueous solution of NaHCO3 and Na2CO3" by Christophe Wylock, Sam Dehaeck, Alexey Rednikov, and Pierre Colinet in Microgravity -- Science and Technology, Volume 20, Numbers 3-4 / September 2008. See: http://www.springerlink.com/content/hp7v05773g7q/?p=1133d99b97064c3a81bb1ccd3eef6fa4π=0
The image Carbon Dioxide-Absorbing Aqueous Brine has not been published.
The image Carbon Dioxide-Absorbing Aqueous Brine cocktail image: not yet published in this form
A similar image (the base image without the cocktail-glass) was published in "A Mach-Zehnder interferometer-based study of evaporation of binary mixtures in Hele-Shaw cells" by S. Dehaeck, Ch. Wylock, and P. Colinet, XXIII International Symposium on Flow Visualisation ISFV13 – XXII French Congress FLUVISU12 (Nice, France, 1-4/07/2008)